It was May 2013, and the Kepler space telescope was dead.
The Kepler planet-hunting mission had been discovering new planets since its launch in 2009, but in May 2013 the second of its four reaction wheels failed. The telescope could no longer control where it pointed; the prime Kepler mission was over.
At the time, I was a third-year undergraduate student at the California Institute of Technology, and it seemed to me that the death of the Kepler mission also signified the death of the goals I had spent the last three years developing. I wanted to study new exoplanet systems and determine what they can tell us about how their planets formed. It seemed to be a great time to be starting in the field—the Kepler space telescope had ushered in a new era of exoplanet discovery, and new planets kept pouring in. The possibilities and opportunities felt endless. Eventually, I was sure, all these discoveries would lead to a unified theory of planet formation, and I wanted to help solve that puzzle using the pieces Kepler was finding.
On supporting science journalism
If you're enjoying this article, consider supporting our award-winning journalism by subscribing. By purchasing a subscription you are helping to ensure the future of impactful stories about the discoveries and ideas shaping our world today.
But without Kepler, the stream of discoveries that had seemed endless only months before seemed to evaporate. I was devastated.
I wouldn’t know for several more years that I was completely wrong about what the death of Kepler meant. The broken telescope was not an end but a new beginning, and it would lead to a transformation in our understanding of a fascinating and mysterious category of planets called hot Jupiters.
The story of hot Jupiters began decades ago. Even before astronomers had developed the technology to be able to look for planets around other stars, scientists imagined what those planets might be like. In 1952 Ukraine-born American astronomer Otto Struve published a short paper suggesting that planets the mass of Jupiter could theoretically reside 50 times closer to their host star than Earth is to the sun. If they did reside so close to their star, he mused, then they should be discoverable in one of two ways: either by monitoring the motion of the host star and looking for its slight reflexive velocity caused by the gravitational tug of an orbiting planet; or by monitoring the star’s brightness and looking for dips in starlight that would occur when a planet passed in front of the star. These two methods, referred to as the radial velocity and transit techniques, respectively, could both theoretically find Jupiter-mass planets orbiting near their star. At the time Struve made this prediction, no one had the technology to test the idea.
But scientists were skeptical, both of the idea that Jupiter-size planets might orbit so close to their sun and the notion that they’d ever be able to detect exoplanets at all. Such large planets, prevailing theories explained, would form farther away, past a point in their planetary system called the ice line, where the growing planet could pull in mass from a large reservoir of icy disk material. As a result, most scientists expected exoplanets to look like the planets of our own solar system: small terrestrial planets would be interior to the ice line and large gas giants would be located beyond it. If this setup prevailed, then astronomers would have to gather data for years or even decades before they could make a discovery. Jupiter orbits the sun once every 12 years, so researchers might have to wait decades to see a Jupiter-like exoplanet move in front of its star. Though theoretically possible, this would be a Herculean task—and smaller terrestrial planets might provide signals too faint to detect.
This understanding shifted entirely in 1995, 43 years after Struve first pondered the possibility of alien Jupiters. That was when astronomers Michel Mayor and Didier Queloz announced the discovery via the radial velocity method of the first exoplanet to be found orbiting a regular star: 51 Pegasi b. This discovery would earn them the Nobel Prize in 2019. A planet about half the mass of Jupiter, 51 Peg b took only 4.2 days to complete one full orbit, and its orbit was only 10 times larger in diameter than the radius of its host star. This extremely short orbital period meant that previous concerns that decades would be needed to confirm an exoplanet orbit suddenly vanished. This planet’s unique combination of being extremely close to its star (and having a very high temperature as a result) and having a large mass (as big as Jupiter, the largest solar system planet) resulted in a signal strong enough to be detected by Mayor and Queloz, as well as a new nickname for this type of planet: “hot Jupiter.” This exoplanet looked like no planet that had ever been seen before, and its size and orbital distance placed it well outside the bounds of classic planet-formation theories.
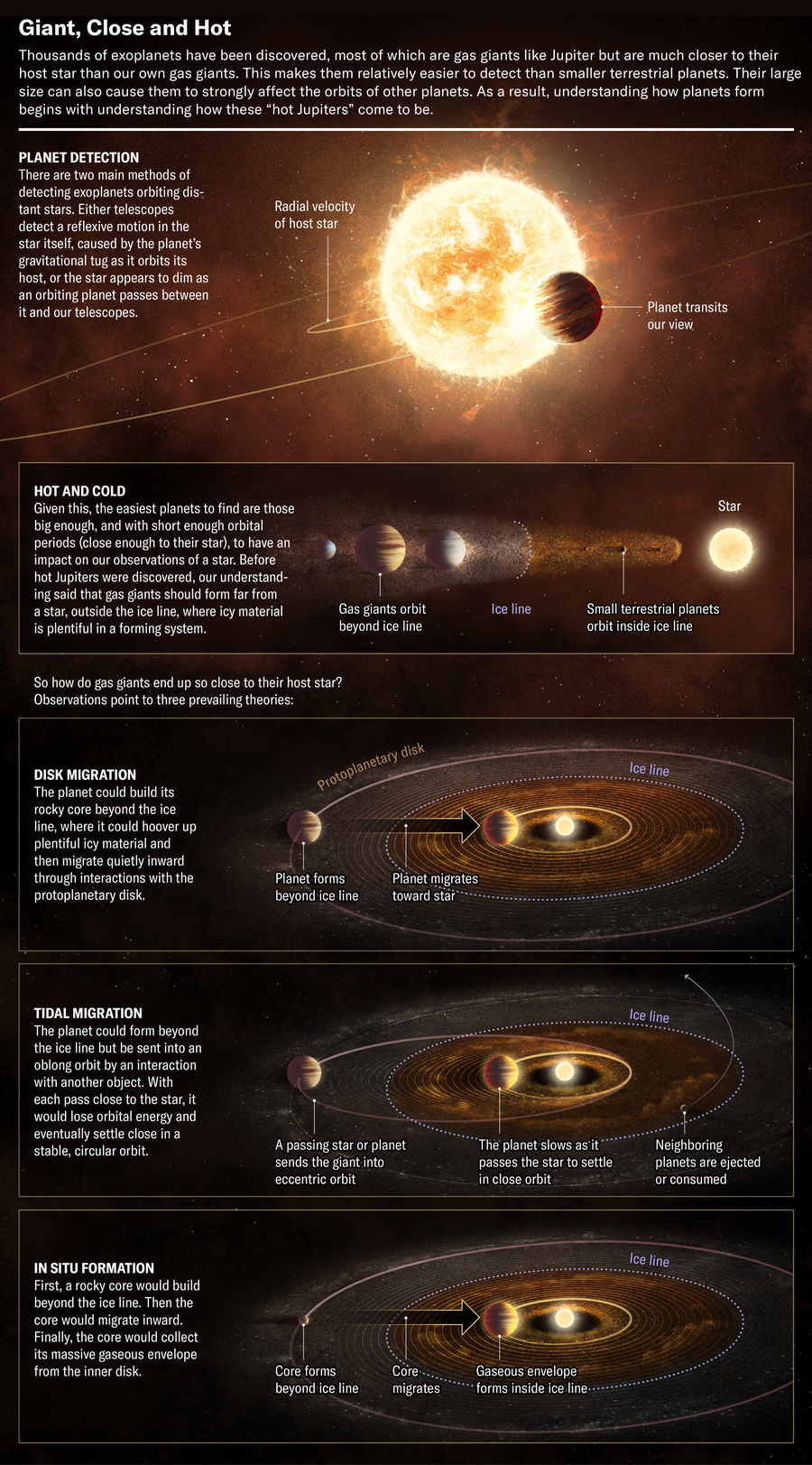
Credit: Matthew Twombly
Immediately scientists began developing new theories on how this kind of planet could have formed. Planets the mass of Jupiter are significantly more massive than all other classes of planets and require a unique process to form. Jupiter-mass planets must first build a core out of rock and ice and then build a gaseous envelope large enough to start a process called runaway accretion, where they hoover up all nearby material and increase their mass 10-fold in less than a million years (a very short time compared to the lifetimes of planetary systems). Classic formation theories predicted that this process would take place far from the star, past the location where the ambient temperature is below the freezing point of water. Less than a year after the initial discovery of 51 Peg b, Doug Lin proposed a mechanism that could create hot Jupiters: Lin suggested that the planet formed past the ice line and then migrated inward after interactions with the protoplanetary disk—the material leftover after the star formed, from which planets are born. The timing of this mechanism made logical sense because Jupiter-sized planets would need to grow to their full girth while the disk is still present and then could make their way inward very efficiently after they were fully formed. This theory of dynamically quiet planet migration, called disk migration, unified the existence of this first hot Jupiter with traditional theories of planet formation because in this scenario, hot Jupiters would form identically to their cold counterparts but would simply move later. Yet the mystery turned out to be a bit more complicated.
After the first planet was found, the race was on to discover more.
After the year 2000, a series of ground-based telescope networks began to emerge, designed to search systematically for more planets like 51 Peg b and another hot Jupiter found early on, HD 209458 b. Soon hot Jupiters became the most plentiful type of planet known by far, though scientists understood that this was because of the ease of finding them and did not necessarily reflect how prevalent they truly were. The big, massive planets orbiting so close to their star gave particularly large signals both in radial velocities (because a massive, close-in planet will tug the star around more) and in transits (because a close-in planet has a higher probability of passing in front of the star from our line of sight and will block out more starlight because of its larger radius).
As time passed, scientists discovered dozens and then hundreds of planets that fit a relatively narrow profile: they had masses and radii similar to Jupiter’s, orbital periods of a few days and orbits that were perfectly or nearly perfectly circular. The similarity of these planets was remarkable, and it seemed to indicate that whatever processes produced these planets were similar across the variety of systems studied. The collection of known hot Jupiters, however, was limited by ground-based observational capabilities, which left open the possibility of many undiscovered aspects of these systems that would require alternative observational techniques to uncover.
Enter the Kepler space telescope. Launched in 2009, the observatory immediately changed the field. Whereas ground-based observations were limited to only the largest planets orbiting stars, Kepler’s precision could uncover much smaller planets. Soon after launch Kepler found its first multiplanet system, Kepler-9, in which two planets orbited the same star. Soon it had discovered hundreds and then thousands of planets.
By this point scientists had spotted so many hot Jupiters that large-scale demographics and population studies were starting to become possible. Initial estimates found that hot Jupiters likely orbited 0.1 percent of stars.
Multiplanet systems seemed to dominate in general, although a 2012 study used the available Kepler data to conclude that hot Jupiters tended not to have planetary siblings. There appeared to be something unique about hot Jupiters, providing a stark contrast to the picture that was developing of exoplanets in general.
A second, more recent theory of hot Jupiter formation called tidal migration could potentially explain the lack of these companions. In this newer, more violent, mechanism, a Jupiter-mass planet forms past the ice line in its system. After time has passed and the protoplanetary disk has dissipated, an interaction between the planet and another object (such as a passing star or another planet) causes the Jupiter’s orbit to become eccentric, or oblong. With an extremely eccentric orbit, the Jupiter will come close to its host star once per orbit. Each time it passes close to its star, the Jupiter will lose some of its orbital energy, making its orbit shrink and return to a circular shape over time. This process would be very destabilizing to any other planets within the Jupiter’s initial orbit, potentially evicting them from the planetary system, and would therefore naturally explain the lack of companions to hot Jupiters seen in the Kepler data. Because of its consistency with the new data, tidal migration became the preferred theory for hot Jupiter formation, supplanting the theory of disk migration first proposed to explain 51 Peg b.
All this success from Kepler shows why I and so many other scientists were dismayed when one of Kepler’s reaction wheels failed in July 2012 and another wheel failure followed in May 2013.
At first it seemed that all hope was lost, but following nearly a year of uncertainty, the Kepler team figured out a new way to operate the telescope that cleverly balanced the spacecraft using the pressure of stellar radiation. In its new incarnation, christened K2, the telescope could continue its search for exoplanets by surveying a new selection of stars every 90 days. Although the shorter observation baselines provided some limitations, the new observational fields also provided high-quality data on an almost unmanageable number of planet hosts. Every 90 days data from a different section of the sky would become available.
Every time a new field was downloaded from the spacecraft, I exchanged excited messages with my fellow researchers. Less than a year after the new mission began, K2 observed a field containing a star called WASP-47, the host of an apparently typical hot Jupiter discovered earlier. In July 2015, I exchanged messages with astronomer Andrew Vanderburg about a series of brand-new signals suggesting additional planets might be transiting in front of the star. Vanderburg had learned about the signals from Hans Martin Schwengeler, an amateur scientist in Switzerland. These new transits stopped me in my tracks. WASP-47 was a known hot Jupiter host—but the K2 light curve showed not just the one expected planet but two additional nearby planets as well. One of them was a Neptune-size planet orbiting just slightly exterior to the known hot Jupiter, and the other was a bit larger than Earth, orbiting interior to the hot Jupiter’s path.
For a moment, it was like my own reaction wheels had failed as my mind spun with the implications. Remember, the consensus in the field at that time was that hot Jupiters never had nearby planetary companions. Yet this beautiful system was a counterexample to that rule. If this hot Jupiter had migrated, its internal and external companions had both survived its journey!
In August 2015 we published our discovery of the new planets, including an analysis where we showed that even if the planets had not happened to transit the star, we still could have discovered one of them just by the gravitational tug it would exert on the hot Jupiter. Less than two days after announcing the planets publicly, I received an e-mail from French scientist Marion Neveu-VanMalle. She explained that her team had been monitoring the WASP-47 system using the radial velocity method and that they had found an additional fourth planet in the system—a cold Jupiter-mass planet, WASP-47 c, orbiting distantly from the inner three planets. Whatever migration process might have occurred must have allowed both the inner companions to the hot Jupiter to survive and also allowed the cold Jupiter companion to stay put in its distant orbit.
This discovery, combined with our discoveries and the original work by the WASP team, meant we now knew that the WASP-47 system contained an unprecedented geometry: it was the only hot Jupiter known to have nearby planetary companions, and there was a distant, colder Jupiter in the system as well. This setup showed that there was something lacking in our theories of hot Jupiter formation.
In 2016 astronomers Konstantin Batygin, Peter Bodenheimer and Greg Laughlin published a paper that suggested a third theory of how hot Jupiters come to be: a piecemeal mechanism they called in situ formation. In this scenario, a hot Jupiter forms in three steps. First, its rocky core builds up past the ice line out of cold disk material; then the core migrates inwards toward its final hot orbit; finally, the core accretes its massive gaseous envelope from disk material streaming by its location. Notably, this theory did predict that exterior companions to hot Jupiters should exist, which was consistent with the newly discovered planets in WASP-47.
After the WASP-47 surprise, astronomers were galvanized to look for more rule-breaking systems.
The next big step forward came following the 2018 launch of the Transiting Exoplanet Survey Satellite (TESS), which is designed to search the brightest stars across the entire sky for exoplanets. In 2020 I worked on a team led by astronomer Chelsea Huang to examine a star called TOI-1130 using TESS observations. We found that the system hosted a hot Jupiter with an interior planetary companion. In addition to that system, other teams found three more hot Jupiters with companions, bringing the total number of systems containing hot Jupiters with siblings to five.
These five systems, combined with the hundreds of systems where a hot Jupiter is known but there is no evidence of additional nearby planets, leave all three of the major formation theories as options: dynamically quiet disk migration, violent and destabilizing tidal migration and piecemeal in situ formation. Each of these three mechanisms seems feasible for a subset of the known population of hot Jupiters. Could it be that all three scenarios sometimes occur?
Despite initial impressions that hot Jupiters were a remarkably uniform population of planets with similar properties, it now seems likely that hot Jupiters with companions formed in a different way than the lonely hot Jupiters. During the next several years TESS should continue to find more systems containing hot Jupiters with companions, and the location and properties of those companions will help paint a more complete picture of the possible architectures of hot Jupiter systems.
The next step in fully understanding hot Jupiters is to use these discoveries to establish the relative likelihoods of the three possible migration mechanisms in order to determine which systems formed which way. Jupiter-sized planets are the rulers of their planetary system because of their dominant gravitational influence and the way their migration pathway sculpts the architectures of their system. Understanding these worlds is the first step to constructing a unified theory of planet formation that scientists have been seeking for centuries.